Engineering a Model to Study Viral Infections: Bioprinting, Microfluidics, and Organoids to Defeat Coronavirus Disease 2019 (COVID-19)
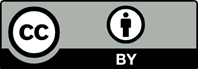
While the number of studies related to severe acute respiratory syndrome-related coronavirus 2 (SARS-CoV-2) is constantly growing, it is essential to provide a framework of modeling viral infections. Therefore, this review aims to describe the background presented by earlier used models for viral studies and an approach to design an “ideal” tissue model for SARS-CoV-2 infection. Due to the previous successful achievements in antiviral research and tissue engineering, combining the emerging techniques such as bioprinting, microfluidics, and organoid formation are considered to be one of the best approaches to form in vitro tissue models. The fabrication of an integrated multi-tissue bioprinted platform tailored for SARSCoV-2 infection can be a great breakthrough that can help defeat coronavirus disease in 2019.
1. Choong YYC, Tan HW, Patel DC, et al., 2020, The Global Rise of 3D Printing During the COVID-19 Pandemic. Nat Rev Mater,1–3. DOI: 10.1038/s41578-020-00234-3.
2. Hossein-Khannazer N, Shokoohian B, Shpichka A, et al., 2020, Novel Therapeutic Approaches for Treatment of COVID-19. J Mol Med, 3:1–15. DOI: 10.1007/s00109-020- 01927-6.
3. Park SJ, Yu KM, Kim YI, et al., 2020, Antiviral Efficacies of FDA-Approved Drugs against SARS-COV-2 Infection in Ferrets. MBio, 11(3):e01114–20. DOI: 10.1128/mbio.01114-20.
4. Shi J, Wen Z, Zhong G, et al., 2020, Susceptibility of Ferrets, Cats, Dogs, and other Domesticated Animals to SARS-coronavirus 2. Science, 368(6494):1016–20. DOI: 10.1126/ science.abb7015.
5. Le Bras A, 2020, SARS-CoV-2 Causes COVID-19-Like Disease in Cynomolgus Macaques. Lab Anim, 49(6):174. DOI: 10.1038/s41684-020-0571-8.
6. Rockx B, Kuiken T, Herfst S, et al., 2020, Comparative Pathogenesis of COVID-19, MERS, and SARS in a Nonhuman Primate Model. Science, 368(6494):1012–5.
7. Lutz C, Maher L, Lee C, et al., 2020, COVID-19 Preclinical Models: Human Angiotensin-Converting Enzyme 2 Transgenic Mice. Hum Genomics, 14:20. DOI: 10.1186/ s40246-020-00272-6.
8. Monteil V, Kwon H, Prado P, et al., 2020, Inhibition of SARS-CoV-2 Infections in Engineered Human Tissues Using Clinical-Grade Soluble Human ACE2. Cell, 181(4):905–13. DOI: 10.1016/j.cell.2020.04.004.
9. Lindesmith L, Moe C, Marionneau S, et al., 2003, Human Susceptibility and Resistance to Norwalk Virus Infection. Nat Med, 9(5):548–53. DOI: 10.1038/nm860.
10. DeVincenzo JP, Wilkinson T, Vaishnaw A, et al., 2010, Viral Load Drives Disease in Humans Experimentally Infected with Respiratory Syncytial Virus. Am J Respir Crit Care Med, 182(10):1305–14. DOI: 10.1164/rccm.201002-0221oc.
11. Memoli MJ, Shaw PA, Han A, et al., 2016, Evaluation of Antihemagglutinin and Antineuraminidase Antibodies as Correlates of Protection in an Influenza A/H1N1 Virus Healthy Human Challenge Model. MBio, 7(2):1–12. DOI: 10.1128/mbio.00417-16.
12. Wilkinson TM, Li CK, Chui CS, et al., 2012, Preexisting Influenza-Specific CD4 + T Cells Correlate with Disease Protection against Influenza Challenge in Humans. Nat Med, 18(2):274–80. DOI: 10.1038/nm.2612.
13. He XS, Holmes TH, Zhang C, et al., 2006, Cellular Immune Responses in Children and Adults Receiving Inactivated or Live Attenuated Influenza Vaccines. J Virol, 80(23):11756– 66. DOI: 10.1128/jvi.01460-06.
14. Huang KY, Li CK, Clutterbuck E, et al., 2014, Virus-Specific Antibody Secreting Cell, Memory B-Cell, and Sero-antibody Responses in the Human Influenza Challenge Model. J Infect Dis, 209(9):1354–61. DOI: 10.1093/infdis/jit650.
15. Larsen CP, Whitehead SS, Durbin AP, 2015, Dengue Human Infection Models to Advance Dengue Vaccine Development. Vaccine, 33(50):7075–82. DOI: 10.1016/j. vaccine.2015.09.052.
16. Kirkpatrick BD, Whitehead SS, Pierce KK, et al., 2016, The Live Attenuated Dengue Vaccine TV003 Elicits Complete Protection Against Dengue in a Human Challenge Model. Sci Transl Med,
8(330): 330ra36. DOI: 10.1126/scitranslmed.aaf1517.
17. Henle W, Henle G, Stokes J Jr., 1943, Demonstration of the Efficacy of Vaccination Against Influenza Type A by Experimental Infection of Human Beings. J Immunol, 46(3):163–75.
18. Jackson GG, Muldon RL, Akers LW, 1963, Serological Evidence for Prevention of Influenzal Infection in Volunteers by an Anti-influenzal Drug Adamantanamine Hydrochloride. Antimicrob Agents Chemother, 161:703–7.
19. Calfee DP, Peng AW, Cass LM, et al., 1999, Safety and Efficacy of Intravenous Zanamivir in Preventing Experimental Human Influenza A Virus Infection. Antimicrob Agents Chemother, 43(7):1616–20. DOI: 10.1128/aac.43.7.1616.
20. Hayden FG, Zylidnikov DM, Iljenko VI, et al., 1982, Comparative Therapeutic Effect of Aerosolized and Oral Rimantadine HC1 in Experimental Human Influenza A Virus Infection. Antiviral Res, 2(3):147–53. DOI: 10.1016/0166- 3542(82)90016-x.
21. Hayden FG, Treanor JJ, Fritz RS, et al., 1999, Use of the Oral Neuraminidase Inhibitor Oseltamivir in Experimental Human Influenza Randomized Controlled Trials for Prevention and Treatment. JAMA, 282(13):1240-6. DOI: 10.1001/ jama.282.13.1240.
22. Mironov V, Visconti R, Kasyanov V, et al., 2009, Organ Printing: Tissue Spheroids as Building Blocks. Biomaterials, 30(12):2164–74. DOI: 10.1016/j.biomaterials.2008.12.084.
23. Gubareva LV, Kaiser L, Matrosovich MN, et al., 2001, Selection of Influenza Virus Mutants in Experimentally Infected Volunteers Treated with Oseltamivir. J Infect Dis, 183(4):523–31. DOI: 10.1086/318537.
24. McCallin S, Sarker SA, Sultana S, et al., 2018, Metagenome Analysis of Russian and Georgian Pyophage Cocktails and a Placebo-Controlled Safety Trial of Single Phage Versus Phage Cocktail in Healthy Staphylococcus aureus Carriers. Environ Microbiol, 20(9):3278–93. DOI: 10.1111/1462-2920.14310.
25. Jault P, Leclerc T, Jennes S, et al., 2019, Efficacy and Tolerability of a Cocktail of Bacteriophages to Treat Burn Wounds Infected by Pseudomonas aeruginosa (PhagoBurn): A Randomised, Controlled, Double-Blind Phase 1/2 Trial. Lancet Infect Dis, 19(1):35–45. DOI: 10.1016/s1473- 3099(18)30482-1.
26. Febvre HP, Rao S, Gindin M, et al., 2019, PHAGE Study: Effects of Supplemental Bacteriophage Intake on Inflammation and Gut Microbiota in Healthy Adults. Nutrients, 11(3):666. DOI: 10.3390/nu11030666.
27. Bem RA, Domachowske JB, Rosenberg HF, 2011, Animal Models of Human Respiratory Syncytial Virus Disease. Am J Physiol Lung Cell Mol Physiol, 301:L148-56.
28. Radigan KA, Misharin AV, Chi M, et al., 2015, Modeling Human Influenza Infection in the Laboratory. Infect Drug Resist, 8:311–20. DOI: 10.2147/idr.s58551.
29. Dowall SD, Graham VA, Rayner E, et al. 2016, A Susceptible Mouse Model for Zika Virus Infection. PLoS Negl Trop Dis, 10(5):e0004658.
30. Ter Meulen J, Bakker AB, Van Den Brink EN, et al., 2004, Human Monoclonal Antibody as Prophylaxis for SARS Coronavirus Infection in Ferrets. Lancet, 363(9427):2139– 41. DOI: 10.1016/s0140-6736(04)16506-9.
31. Ng PS, Böhm R, Hartley-Tassell LE, et al., 2014, Ferrets Exclusively Synthesize Neu5Ac and Express Naturally Humanized Influenza A Virus Receptors. Nat Commun, 5(1):1–9. DOI: 10.1038/ncomms6750.
32. Hancock GE, Smith JD, Heers KM, 2000, Serum Neutralizing Antibody Titers of Seropositive Chimpanzees Immunized with Vaccines Coformulated with Natural Fusion and Attachment Proteins of Respiratory Syncytial Virus. J Infect Dis, 181(5):1768–71. DOI: 10.1086/315475.
33. Clarke CJ, Watt NJ, Meredith A, et al., 1994, Respiratory Syncytial Virus-associated Bronchopneumonia in a Young Chimpanzee. J Comp Pathol, 110(2):207–12. DOI: 10.1016/ s0021-9975(08)80191-0.
34. Perrin S, 2014, Make Mouse Studies Work. Nature, 517:423–5.
35. Ito R, Takahashi T, Ito M, 2018, Humanized Mouse Models: Application to Human Diseases. J Cell Physiol, 233:3723–8. DOI: 10.1002/jcp.26045.
36. Walsh NC, Kenney LL, Jangalwe S, et al., 2017, Humanized Mouse Models of Clinical Disease. Annu Rev Pathol Mech Dis, 12(1):187–215. DOI: 10.1146/annurev-pathol-052016-100332.
37. Kistner O, Barrett PN, Mundt W, et al., 1998, Development of a Mammalian Cell (Vero) Derived Candidate Influenza Virus Vaccine. Vaccine, 16(9-10):960–8. DOI: 10.1016/ s0264-410x(97)00301-0.
38. de Lang A, Osterhaus AD, Haagmans BL, 2006, Interferon-γ and Interleukin-4 Downregulate Expression of the SARS Coronavirus Receptor ACE2 in Vero E6 Cells. Virology, 353(2):474–81. DOI: 10.1016/j.virol.2006.06.011.
39. Bhowmick R, Derakhshan T, Liang Y, et al., 2018, A Three- Dimensional Human Tissue-Engineered Lung Model to Study Influenza A Infection. Tissue Eng Part A, 24(19-20):1468–80. DOI: 10.1089/ten.tea.2017.0449.
40. Dill V, Hoffmann B, Zimmer A, et al., 2018, Influence of Cell Type and Cell Culture Media on the Propagation of Foot-and- Mouth Disease Virus with Regard to Vaccine Quality. Virol J, 15(1):1–11. DOI: 10.1186/s12985-018-0956-0.
41. Radlett PJ, Pay TW, Garland AJ, et al., 1985, The Use of BHK Suspension Cells for the Commercial Production of Foot and Mouth Disease Vaccines Over a Twenty Year Period. Dev Biol Stand, 60:163–70.
42. Archer F, Jacquier E, Lyon M, et al., 2007, Alveolar Type II Cells Isolated from Pulmonary Adenocarcinoma: A Model for JSRV Expression In Vitro. Am J Respir Cell Mol Biol, 36(5):534–40. DOI: 10.1165/rcmb.2006-0285oc.
43. Hayle AJ, 1986, Culture of Respiratory Syncytial Virus Infected Diploid Bovine Nasal Mucosa Cells on Cytodex 3 Microcarriers. Arch Virol, 89(1–4):81–8. DOI: 10.1007/ bf01309881.
44. Gardner JK, Herbst-Kralovetz MM, 2016, Three- Dimensional Rotating Wall Vessel-Derived Cell Culture Models for Studying Virus-Host Interactions. Viruses, 8:304. DOI: 10.3390/v8110304.
45. Sachs N, Papaspyropoulos A, Zomer-van Ommen DD, et al., 2019, Long-term Expanding Human Airway Organoids for Disease Modeling. EMBO J, 38(4):e100300.
46. Chen YW, Huang SX, De Carvalho AL, et al., 2017, A Three-dimensional Model of Human Lung Development and Disease from Pluripotent Stem Cells. Nat Cell Biol, 19(5):542–9.
47. Zhou J, Li C, Sachs N, et al., 2018, Differentiated Human Airway Organoids to Assess Infectivity of Emerging Influenza Virus. Proc Natl Acad Sci USA, 115(26):6822–7. DOI: 10.1073/pnas.1806308115.
48. Ettayebi K, Crawford SE, Murakami K, et al., 2016, Replication of Human Noroviruses in Stem Cell-derived Human Enteroids. Science, 353(6306):1387–93.
49. Zhou J, Li C, Zhao G, et al., 2017, Human Intestinal Tract Serves as an Alternative Infection Route for Middle East Respiratory Syndrome Coronavirus. Sci Adv, 3(11):eaao4966.
50. Grivel JC, Margolis L, 2009, Use of Human Tissue Explants to Study Human Infectious Agents. Nat Protoc, 4(2):256–69.
51. Koban R, Neumann M, Daugs A, et al., 2018, A Novel Three-dimensional Cell Culture Method Enhances Antiviral Drug Screening in Primary Human Cells. Antiviral Res, 150:20–9. DOI: 10.1016/j.antiviral.2017.12.005.
52. Imle A, Kumberger P, Schnellbächer ND, et al., 2019, Experimental and Computational Analyses Reveal that Environmental Restrictions Shape HIV-1 Spread in 3D Cultures. Nat Commun, 10(1):2144. DOI: 10.1038/s41467- 019-09879-3.
53. Shokoohian B, 2018, Bio-printing Damaged Tissues: A Novel Approach in Regenerative Medicine. Mod Med Lab J, 2(1):1–5. DOI: 10.30699/mmlj17.2.1.1.
54. Berg J, Hiller T, Kissner MS, et al., Optimization of Cell-laden Bioinks for 3D Bioprinting and Efficient Infection with Influenza A Virus. Sci Rep, 8(1):1–13. DOI: 10.1038/s41598- 018-31880-x.
55. Park JA, Yoon S, Kwon J, et al., 2017, Freeform Micropatterning of Living Cells into Cell Culture Medium Using Direct Inkjet Printing. Sci Rep, 7(1):14610. DOI: 10.1038/s41598-017-14726-w.
56. Du G, Fang Q, den Toonder JM, 2016, Microfluidics for Cell- Based High Throughput Screening Platforms a Review. Anal Chim Acta, 903:36–50. DOI: 10.1016/j.aca.2015.11.023.
57. Montanez-Sauri SI, Sung KE, Puccinelli JP, et al., 2011, Automation of Three-Dimensional Cell Culture in Arrayed Microfluidic Devices. J Lab Autom, 16(3):171–85. DOI: 10.1016/j.jala.2011.02.003.
58. Kane KI, Moreno EL, Hachi S, et al., 2019, Automated Microfluidic Cell Culture of Stem Cell Derived Dopaminergic Neurons. Sci Rep, 9(1):1–12.
59. Super A, Jaccard N, Marques MP, et al., 2016, Real- Time Monitoring of Specific Oxygen Uptake Rates of Embryonic Stem Cells in a Microfluidic Cell Culture Device. Biotechnol J, 11(9):1179–89. DOI: 10.1002/biot.201500479.
60. Vergani M, Carminati M, Ferrari G, et al., 2012, Multichannel Bipotentiostat Integrated with a Microfluidic Platform for Electrochemical Real-time Monitoring Of Cell Cultures. IEEE Trans Biomed Circuits Syst, 6(5):498–507. DOI: 10.1109/tbcas.2012.2187783.
61. Yum K, Hong SG, Healy KE, et al., 2013, Physiologically Relevant Organs on Chips. Biotechnol J, 9(1):16–27. DOI: 10.1002/biot.201300187.
62. Costello DA, Millet JK, Hsia CY, et al., 2013, Single Particle Assay of Coronavirus Membrane Fusion with Proteinaceous Receptor-embedded Supported Bilayers. Biomaterials, 34(32):7895–904. DOI: 10.1016/j.biomaterials.2013.06.034.
63. Poortahmasebi V, Baghi HB, 2019, Living in the Shadows of Hepatitis. Lancet Infect Dis, 19(11):1171–2. DOI: 10.1016/ s1473-3099(19)30534-1.
64. Ringehan M, McKeating JA, Protzer U, 2017, Viral Hepatitis and Liver Cancer. Philos Trans R Soc Lond B Biol Sci, 372:20160274. DOI: 10.1098/rstb.2016.0274.
65. Chong TW, Smith RL, Hughes MG, et al., 2006, Primary Human Hepatocytes in Spheroid Formation to Study Hepatitis C Infection. J Surg Res, 130(1):52–7.
66. Nie YZ, Zheng YW, Miyakawa K, et al., 2008, Recapitulation of Hepatitis B Virus Host Interactions in Liver Organoids from Human Induced Pluripotent Stem Cells. EBioMedicine, 35:114–23. DOI: 10.1016/j.ebiom.2018.08.014.
67. Tajpara P, Mildner M, Schmidt R, et al., 2019, A Preclinical Model for Studying Herpes Simplex Virus Infection. J Invest Dermatol, 139:673–82.
68. Zhu Y, Yang Y, Guo J, et al., 2017, Ex vivo 2D and 3D HSV-2 Infection Model Using Human Normal Vaginal Epithelial Cells. Oncotarget, 8(9):15267–82. DOI: 10.18632/oncotarget.14840.
69. D’Aiuto L, Naciri J, Radio N, et al., 2018, Generation of Three-dimensional Human Neuronal Cultures: Application to Modeling CNS Viral Infections. Stem Cell Res Ther, 9(1):134. DOI: 10.1186/s13287-018-0881-6.
70. Sison SL, O’Brien BS, Johnson AJ, et al., 2019, Human Cytomegalovirus Disruption of Calcium Signaling in Neural Progenitor Cells and Organoids. J Virol, 93(17):954. DOI: 10.1128/jvi.00954-19.
71. Berg J, Hiller T, Kissner MS, et al., 2018, Optimization of Cell-laden Bioinks for 3D Bioprinting and Efficient Infection with Influenza A Virus. Sci Rep, 8(1):13877. DOI: 10.1038/ s41598-018-31880-x.
72. Sainz B, Tencate V, Uprichard SL, 2009, Three-Dimensional Huh7 Cell Culture System for the Study of Hepatitis C Virus Infection. Virol J, 6:103. DOI: 10.1186/1743-422x-6-103.
73. Molina-Jimenez F, Benedicto I, Thi VL, et al., 2012, Matrigel- Embedded 3D Culture of Huh-7 Cells as a Hepatocyte-Like Polarized System to Study Hepatitis C Virus Cycle. Virology, 425(1):31–9. DOI: 10.1016/j.virol.2011.12.021.
74. Rajalakshmy AR, Malathi J, Madhavan HN, et al., 2015, Mebiolgel, a Thermoreversible Polymer as a Scaffold for Three Dimensional Culture of Huh7 Cell Line with Improved Hepatocyte Differentiation Marker Expression and HCV Replication. Indian J Med Microbiol, 33(4):554–9. DOI: 10.4103/0255-0857.167330.
75. Ortega-Prieto AM, Skelton JK, Wai SN, et al., 2018, 3D Microfluidic Liver Cultures as a Physiological Preclinical Tool for Hepatitis B Virus Infection. Nat Commun, 9(1):682. DOI: 10.1038/s41467-018-02969-8.
76. Morrison KM, Beucler MJ, Campbell EO, et al., 2018, Development of a Primary Human Cell Model for the Study of Human Cytomegalovirus Replication and Spread within Salivary Epithelium. J Virol, 93(3):e01608–18. DOI: 10.1128/jvi.01608-18.
77. Lam V, Bigley T, Terhune SS, et al., 2012, A Method for Quantifying Mechanical Properties of Tissue Following Viral Infection. PLoS One, 7(8):e42197. DOI: 10.1371/journal. pone.0042197.
78. Goodwin TJ, McCarthy M, Osterrieder N, et al., 2013, Three- Dimensional Normal Human Neural Progenitor Tissue-Like Assemblies: A Model of Persistent Varicella-Zoster Virus Infection. PLoS Pathog, 9(8):e1003512. DOI: 10.1371/ journal.ppat.1003512.
79. Li BJ, Tang Q, Cheng D, et al., 2005, Using siRNA in Prophylactic and Therapeutic Regimens against SARS Coronavirus in Rhesus Macaque. Nat Med, 11(9):944–51. DOI: 10.1038/nm1280.
80. Jeon S, Ko M, Lee J, et al., 2020, Identification of Antiviral Drug Candidates against SARS-CoV-2 from FDA-approved Drugs. Antimicrob Agents Chemother, 64(7):e00819-20. DOI: 10.1128/aac.00819-20.
81. Ziegler CG, Allon SJ, Nyquist SK, et al., 2020, SARS-CoV-2 Receptor ACE2 is an Interferon-Stimulated Gene in Human Airway Epithelial Cells and is Detected in Specific Cell Subsets across Tissues. Cell, 181(5):1016–35. DOI: 10.3410/f.737831436.793574366.
82. Li W, Moore MJ, Vasilieva N, et al., 2003, Angiotensin- Converting Enzyme 2 is a Functional Receptor for the SARS Coronavirus. Nature, 426(6965):450–4. DOI: 10.1038/ nature02145.
83. Glowacka I, Bertram S, Muller MA, et al., 2011, Evidence that TMPRSS2 Activates the Severe Acute Respiratory Syndrome Coronavirus Spike Protein for Membrane Fusion and Reduces Viral Control by the Humoral Immune Response. J Virol, 85(9):4122–34. DOI: 10.1128/jvi.02232-10.
84. Li F, Li W, Farzan M, et al., 2005, Structural Biology: Structure of SARS Coronavirus Spike Receptor-binding Domain Complexed with Receptor. Science, 309(5742):1864–8. DOI: 10.1126/science.1116480.
85. Shirato K, Kawase M, Matsuyama S, 2013, Middle East Respiratory Syndrome Coronavirus Infection Mediated by the Transmembrane Serine Protease TMPRSS2. J Virol, 87(23):12552–61. DOI: 10.1128/jvi.01890-13.
86. Hoffmann M, Kleine-Weber H, Schroeder S, et al., 2020, SARS-CoV-2 Cell Entry Depends on ACE2 and TMPRSS2 and Is Blocked by a Clinically Proven Protease Inhibitor. Cell, 181(2):271–280. DOI: 10.1016/j.cell.2020.02.052.
87. Zang R, Castro MF, McCune BT, et al., 2020, TMPRSS2 and TMPRSS4 Promote SARS-CoV-2 Infection of Human Small Intestinal Enterocytes. Sci Immunol, 5(47):1–15. DOI: 10.1126/sciimmunol.abc3582.
88. Hamming I, Timens W, Bulthuis ML, et al., 2004, Tissue Distribution of ACE2 Protein, the Functional Receptor for SARS Coronavirus. A First Step in Understanding SARS Pathogenesis. J Pathol, 203(2):631–7. DOI: 10.1002/path.1570.
89. Bilinska K, Jakubowska P, Von Bartheld CS, et al., 2020, Expression of the SARS-CoV-2 Entry Proteins, ACE2 and TMPRSS2, in Cells of the Olfactory Epithelium: Identification of Cell Types and Trends with Age. ACS Chem Neurosci, 11(11):1555–62. DOI: 10.1021/acschemneuro.0c00210.
90. Harmer D, Gilbert M, Borman R, et al., 2002, Quantitative mRNA Expression Profiling of ACE 2, a Novel Homologue of Angiotensin Converting Enzyme. FEBS Lett, 532(1– 2):107–10. DOI: 10.1016/s0014-5793(02)03640-2.
91. Wang Z, Xu X, 2020, scRNA-seq Profiling of Human Testes Reveals the Presence of the ACE2 Receptor, A Target for SARS-CoV-2 Infection in Spermatogonia, Leydig and Sertoli Cells. Cells, 9:920. DOI: 10.3390/cells9040920.
92. Song H, Seddighzadeh B, Cooperberg MR, et al., 2020, Expression of ACE2, the SARS-CoV-2 Receptor, and TMPRSS2 in Prostate Epithelial Cells. Eur Urol, 78(2):296– 8. DOI: 10.1101/2020.04.24.056259.
93. Qi F, Qian S, Zhang S, et al., 2020, Single Cell RNA Sequencing of 13 Human Tissues Identify Cell Types and Receptors of Human Coronaviruses. Biochem Biophys Res Commun, 526(1):135–40. DOI: 10.1101/2020.02.16.951913.
94. Zou X, Chen K, Zou J, et al., 2020, Single-Cell RNA-seq Data Analysis on the Receptor ACE2 Expression Reveals the Potential Risk of Different Human Organs Vulnerable to 2019-nCoV Infection. Front Med, 14(2):185–92. DOI: 10.1007/s11684-020-0754-0.
95. Lukassen S, Chua RL, Trefzer T, et al., 2020, SARS -CoV-2 Receptor ACE 2 and TMPRSS 2 are Primarily Expressed in Bronchial Transient Secretory Cells. EMBO J, 39(10):1–15. DOI: 10.15252/embj.20105114.
96. Chen YW, Lee MS, Lucht A, et al., 2010, TMPRSS2, a Serine Protease Expressed in the Prostate on the Apical Surface of Luminal Epithelial Cells and Released into Semen in Prostasomes, is Misregulated in Prostate Cancer Cells. Am J Pathol, 176(6):2986–96. DOI: 10.2353/ajpath.2010.090665.
97. Sungnak W, Huang N, Bécavin C, et al., 2020, SARS-CoV-2 Entry Factors are Highly Expressed in Nasal Epithelial Cells Together with Innate Immune Genes. Nat Med, 26(5):681–7. DOI: 10.1038/s41591-020-0868-6.
98. Ng WL, Chua CK, Shen YF, 2019, Print Me An Organ! Why We Are Not There Yet. Prog Polym Sci, 97:101145. DOI: 10.1016/j.progpolymsci.2019.101145.
99. Ozbolat IT, Hospodiuk M, 2016, Current Advances and Future Perspectives in Extrusion-Based Bioprinting. Biomaterials,;76:321–43. DOI: 10.1016/j. biomaterials.2015.10.076.
100. Saunders RE, Derby B, 2014, Inkjet Printing Biomaterials for Tissue Engineering: Bioprinting. Int Mater Rev, 59(8):430– 48. DOI: 10.1179/1743280414y.0000000040.
101. Antoshin AA, Churbanov SN, Minaev NV, et al., 2019, LIFT-Bioprinting, is it Worth it? Bioprinting, 15:e00052. DOI: 10.1016/j.bprint.2019.e00052.
102. Ng WL, Lee JM, Zhou M, et al., 2020, Vat Polymerization- Based Bioprinting Process, Materials, Applications and Regulatory Challenges. Biofabrication, 12(2):22001. DOI: 10.1088/1758-5090/ab6034.
103. Matai I, Kaur G, Seyedsalehi A, et al., 2020, Progress in 3D Bioprinting Technology for Tissue/Organ Regenerative Engineering. Biomaterials, 226:119536. DOI: 10.1016/j. biomaterials.2019.119536.
104. Zurina I, Shpichka A, Saburina I, et al., 2018, 2D/3D Buccal Epithelial Cell Self-Assembling as a Tool for Cell Phenotype Maintenance and Fabrication of Multilayered Epithelial Linings In Vitro. Biomed Mater, 13(5):054104. DOI: 10.1088/1748-605x/aace1c.
105. Moldovan NI, Hibino N, Nakayama K, 2017, Principles of the Kenzan Method for Robotic Cell Spheroid-Based Three- Dimensional Bioprinting. Tissue Eng Part B Rev, 23(3):237– 44. DOI: 10.1089/ten.teb.2016.0322.
106. Gorkun AA, Shpichka AI, Zurina IM, et al., 2018, Angiogenic Potential of Spheroids from Umbilical Cord and Adipose- Derived Multipotent Mesenchymal Stromal Cells within Fibrin Gel. Biomed Mater, 13(4):44108. DOI: 10.1088/1748- 605x/aac22d.
107. Shpichka A, Osipova D, Efremov Y, et al., 2020, Fibrin-based Bioinks: New Tricks from an Old Dog. Int J Bioprinting, 6(3):1–14. DOI: 10.18063/ijb.v6i3.269.
108. Kornev VA, Grebenik EA, Solovieva AB, et al., 2018, Hydrogel-assisted Neuroregeneration Approaches Towards Brain Injury Therapy: A State-of-the-Art Review. Comput Struct Biotechnol J, 16:488–502. DOI: 10.1016/j. csbj.2018.10.011.
109. Shpichka AI, Konarev PV, Efremov YM, et al., 2020, Digging Deeper: Structural Background of PEGylated fi Brin Gels in Cell Migration and lumenogenesis. RSC Adv, 10:4190–200. DOI: 10.1039/c9ra08169k.
110. De Rudder C, Arroyo MC, Lebeer S, et al., 2018, Modelling Upper Respiratory Tract Diseases: Getting Grips on Host-microbe Interactions in Chronic Rhinosinusitis Using In Vitro Technologies. Microbiome, 6(1):75. DOI: 10.1186/s40168- 018-0462-z.
111. Wang L, Shen Y, Li M, et al., 2020, Clinical Manifestations and Evidence of Neurological Involvement in 2019 Novel Coronavirus SARS-CoV-2: A Systematic Review and Meta-analysis. J Neurol, 2020;1–13. DOI: 10.1007/s00415-020- 09974-2.
112. Li ST, Young TH, Huang TW, 2018, Poly (ethylene-co- vinyl alcohol) is a Suitable Substrate for Human Olfactory Neuroepithelial Cell Differentiation In Vitro through a Defined Regulatory Pathway. Acta Biomater, 68:204–13. DOI: 10.1016/j.actbio.2017.12.029.
113. Li ST, Young TH, Wang CT, et al., 2018, Chitosan Films Promote Formation of Olfactory Neurospheres and Differentiation of Olfactory Receptor Neurons. Rhinology, 56(4):336–42. DOI: 10.4193/rhin17.155.
114. Du L, Zou L, Wang Q, et al., 2015, A Novel Biomimetic Olfactory Cell-based Biosensor with DNA-Directed Site- Specific Immobilization of Cells on a Microelectrode Array. Sens Actuators B Chem, 217:186–92. DOI: 10.1016/j. snb.2014.08.054.
115. Skaat H, Ziv-Polat O, Shahar A, et al., 2011, Enhancement of Migration, Growth and Differnatiation of Nasal Olfactory Mucosa Cells by Growth Factor-conjugated Fluorescent-maghemite Nanoparticles. Bioconjugate Chem, 22(12):2600– 10. DOI: 10.1021/bc200454k.
116. Horvath L, Umehara Y, Jud C, et al., 2015, Engineering an In Vitro Air-blood Barrier by 3D Bioprinting. Sci Rep, 5:7974. DOI: 10.1038/srep07974.
117. Grigoryan B, Paulsen SJ, Corbett DC, et al., 2019, Multivascular Networks and Functional Intravascular Topologies within Biocompatible Hydrogels. Science, 364(6439):458–64. DOI: 10.1126/science.aav9750.
118. Lewis KJ, Tibbitt MW, Zhao Y, et al., 2015, In Vitro Model Alveoli from Photodegradable Microsphere Templates. Biomater Sci, 3(6):821–32. DOI: 10.1039/c5bm00034c.
119. Ozbolat IT, Moncal KK, Gudapati H, 2017, Evaluation of Bioprinter Technologies. Addit Manuf, 13:179–200. DOI: 10.1016/j.addma.2016.10.003.
120. Miri AK, Nieto D, Iglesias L, et al., 2018, Microfluidics-Enabled Multimaterial Maskless Stereolithographic Bioprinting. Adv Mater, 30(27):e1800242. DOI: 10.1002/adma.201870201.
121. Maiullari F, Costantini M, Milan M, et al., 2018, A Multi-cellular 3D Bioprinting Approach for Vascularized Heart Tissue Engineering Based on HUVECs and iPSC-Derived Cardiomyocytes. Sci Rep, 8(1):1–15. DOI: 10.1038/s41598- 018-31848-x.
122. Zhang YS, Arneri A, Bersini S, et al., 2016, Bioprinting 3D Microfibrous Scaffolds for Engineering Endothelialized Myocardium and Heart-on-a-chip. Biomaterials, 110:45–59. DOI: 10.1016/j.biomaterials.2016.09.003.
123. Pan XW, Xu D, Zhang H, et al., 2020, Identification of a Potential Mechanism of Acute Kidney Injury During the COVID-19 Outbreak: A Study Based on Single-Cell Transcriptome Analysis. Intensive Care Med, 46:1114-1116. DOI: 10.1007/s00134-020-06026-1.
124. Ronco C, Reis T, 2020, Kidney Involvement in COVID-19 and Rationale for Extracorporeal Therapies. Nat Rev Nephrol, 16(6):308–10. DOI: 10.1038/s41581-020-0284-7.
125. Subramanian B, Rudym D, Cannizzaro C, et al., 2010, Tissue- Engineered Three-Dimensional In Vitro Models for Normal and Diseased Kidney. Tissue Eng Part A, 16(9):2821–31. DOI: 10.1089/ten.tea.2009.0595.
126. Sochol RD, Gupta NR, Bonventre JV, 2016, A Role for 3D Printing in Kidney-on-a-Chip Platforms. Curr Transplant Rep, 3(1):82–92. DOI: 10.1007/s40472-016-0085-x.
127. Homan KA, Kolesky DB, Skylar-Scott MA, et al., 2016, Bioprinting of 3D Convoluted Renal Proximal Tubules on Perfusable Chips. Sci Rep, 6:34845. DOI: 10.1038/ srep34845.
128. Ali M, Kumar A, Yoo JJ, Zahran F, et al., 2019, A Photo- Crosslinkable Kidney ECM-Derived Bioink Accelerates Renal Tissue Formation. Adv Healthc Mater, 8(7):1800992. DOI: 10.1002/adhm.201800992.
129. Chuah JKC, Zink D, 2017, Stem Cell-Derived Kidney Cells and Organoids: Recent Breakthroughs and Emerging Applications. Biotechnol Adv, 35(2):150–67. DOI: 10.1016/j. biotechadv.2016.12.001.
130. King SM, Higgins JW, Nino CR, et al., 2017, 3D Proximal Tubule Tissues Recapitulate Key Aspects of Renal Physiology to Enable Nephrotoxicity Testing. Front Physiol, 8(1):1–18. DOI: 10.3389/fphys.2017.00123.
131. Heydari Z, Najimi M, Mirzaei H, et al., 2020, Tissue Engineering in Liver Regenerative Medicine: Insights into Novel Translational Technologies. Cells, 9(2):304.
132. Yanagi Y, Nakayama K, Taguchi T, et al., 2017, In Vivo and Ex Vivo Methods of Growing a Liver Bud Through Tissue Connection. Sci Rep, 7(1):1–15. DOI: 10.1038/s41598-017- 14542-2.
133. Bhise NS, Manoharan V, Massa S, et al., 2016, A Liver-on-a-Chip Platform with Bioprinted Hepatic Spheroids. Biofabrication, 8(1):14101.
134. Hashimoto T, Perlot T, Rehman A, et al., 2012, ACE2 Links Amino Acid Malnutrition to Microbial Ecology and Intestinal Inflammation. Nature, 487(7408):477–81. DOI: 10.1038/ nature11228.
135. Williams CF, Walton GE, Jiang L, et al., 2015, Comparative Analysis of Intestinal Tract Models. Annu Rev Food Sci Technol, 6(1):329–50. DOI: 10.1146/annurev-food-022814-015429.
136. Madden LR, Nguyen TV, Garcia-Mojica S, et al., 2018, Bioprinted 3D Primary Human Intestinal Tissues Model Aspects of Native Physiology and ADME/Tox Functions. iScience, 2:156–67. DOI: 10.1016/j.isci.2018.03.015.
137. Ma J, Wang Y, Liu J, 2018, Bioprinting of 3D Tissues/Organs Combined with Microfluidics. RSC Adv, 8(39):21712–27. DOI: 10.1039/c8ra03022g.
138. Yu F, Choudhury D, 2019, Microfluidic Bioprinting for Organ-on-a-Chip Models. Drug Discov Today, 24(6):1248– 57. DOI: 10.1016/j.drudis.2019.03.025.
139. Miri AK, Mostafavi E, Khorsandi D, et al., 2019, Bioprinters for Organs-on-Chips. Biofabrication, 11(4):42002. DOI: 10.1088/1758-5090/ab2798.
140. Koroleva A, Deiwick A, Nguyen A, et al., 2016, Hydrogel- Based Microfluidics for Vascular Tissue Engineering. BioNanoMaterials, 17(1–2):19–32. DOI: 10.1515/bnm- 2015-0026.
141. Ashammakhi N, Wesseling-Perry K, Hasan A, et al., 2018, Kidney-on-a-Chip: Untapped Opportunities. Kidney Int, 94(6):1073–86. DOI: 10.1016/j.kint.2018.06.034.
142. Ribas J, Sadeghi H, Manbachi A, et al., 2016, Cardiovascular Organ-on-a-Chip Platforms for Drug Discovery and Development. Appl Vitr Toxicol, 2(2):82–96.
143. Jahromi MA, Abdoli A, Rahmanian M, et al., 2019, Microfluidic Brain-on-a-Chip: Perspectives for Mimicking Neural System Disorders. Mol Neurobiol, 56(12):8489–512. DOI: 10.1007/s12035-019-01653-2.
144. Maschmeyer I, Lorenz AK, Schimek K, et al., 2015, A Four- Organ-Chip for Interconnected Long-Term Co-culture of Human Intestine, Liver, Skin and Kidney Equivalents. Lab Chip, 15(12):2688–99. DOI: 10.1039/c5lc00392j.
145. Vernetti L, Gough A, Baetz N, et al., 2017, Functional Coupling of Human Microphysiology Systems: Intestine, Liver, Kidney Proximal Tubule, Blood-Brain Barrier and Skeletal Muscle. Sci Rep, 7(1):1–14. DOI: 10.1038/srep44517.
146. Skardal A, Murphy SV, Devarasetty M, et al., 2017, Multi- Tissue Interactions in an Integrated Three-Tissue Organ-on-a-Chip Platform. Sci Rep, 7(1):1–16.
147. Ramadan Q, Ting FC, 2016, In Vitro Micro-Physiological Immune-Competent Model of the Human Skin. Lab Chip, 16(10):1899–908. DOI: 10.1039/c6lc00229c.
148. Harrington H, Cato P, Salazar F, et al., 2014, Immunocompetent 3D Model of Human Upper Airway for Disease Modeling and In Vitro Drug Evaluation. Mol Pharm, 11(7):2082–91. DOI: 10.1021/mp5000295.
149. Gopalakrishnan N, Hannam R, Casoni GP, et al., 2015, Infection and Immunity on a Chip: A Compartmentalised Microfluidic Platform to Monitor Immune Cell Behaviour in Real Time. Lab Chip, 15(6):1481–7. DOI: 10.1039/ c4lc01438c.
150. Rosa PM, Gopalakrishnan N, Ibrahim H, et al., 2016, The Intercell Dynamics of T Cells and Dendritic Cells in a Lymph Node-on-a-Chip Flow Device. Lab Chip, 16(19):3728–40. DOI: 10.1039/c6lc00702c.